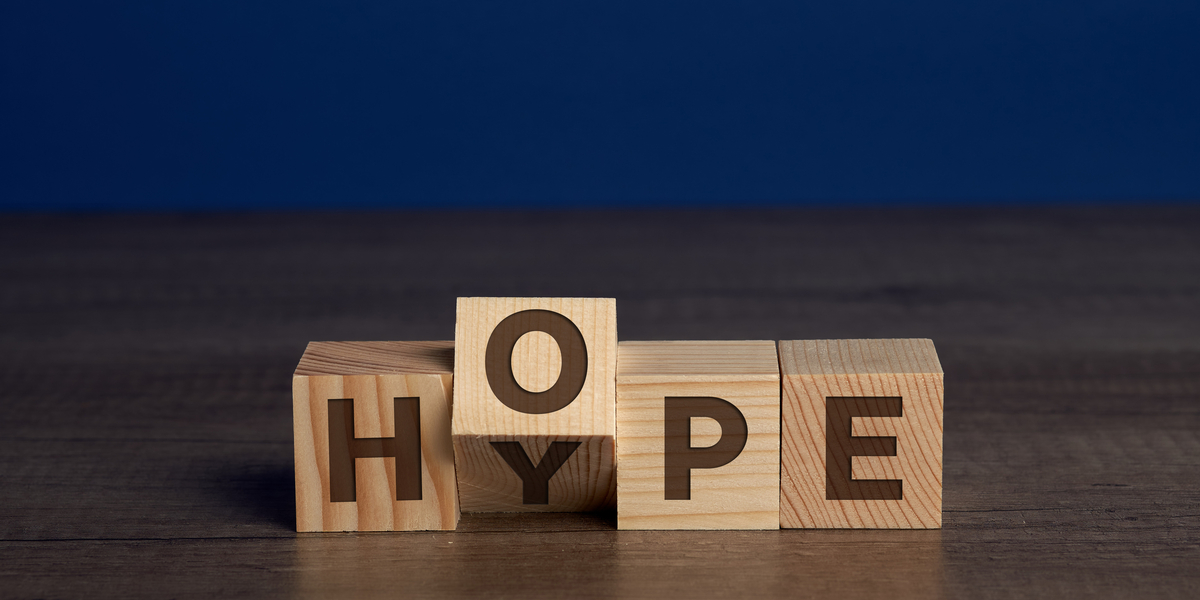
Disclaimer: I have written this piece from a position of privilege – as an HD family member that has been fortunate to receive an education that allows me to deeply understand the nuances of Huntington’s disease. I know what it means not only at the biological level, but also at the family level. I am profoundly aware of the desire for a disease-modifying drug. But my hopes are tempered through the privileged lens of understanding complex scientific data and interpretation. Here, I report facts and my opinion of those facts with no vested interest in any specific therapeutic approach. If Prilenia feels errors have been made, they are invited to reach out and any factual corrections will gladly be made.
Recently, there have been a few press releases from Prilenia Therapeutics about their advancement of the drug pridopidine toward regulatory approval for the treatment of Huntington’s disease (HD). There’s also been mixed messaging about findings from pridopidine clinical trials and statements made by the company. Let’s break down what the research really says and what the recent press releases mean in the bigger picture.
MermaiHD Trial
We’ve written about the long and storied path of pridopidine before. It’s been tested in a number of different clinical trials, by a few different companies. Over the years, the use of pridopidine has shifted. Once thought to have utility for helping to regulate movements associated with HD, it’s now being tested for potentially slowing the disease course.
In 2008, pridopidine (previously called Huntexil) was tested in a European Phase 2 trial called MermaiHD. Back then, the company running the trial, NeuroSearch, thought the drug could be used to help with changes in movement control caused by HD.
Specifically, they thought the drug could help people control their voluntary movements that get more stiff and rigid as HD progresses. While people on pridopidine had slight improvements in movement control, the effect wasn’t large enough to determine that it was caused by pridopidine, and the trial failed to meet its endpoints.
HART Trial
Around the same time, NeuroSearch also carried out the HART study in America. Again in this study, there wasn’t a conclusive improvement in voluntary movements associated with stiffness and rigidity.
After the HART study, they pooled that data with the MermaidHD study to find that with this combined dataset, there did seem to be an improvement on voluntary movements. However, both the US FDA and European EMA determined that a larger trial was needed to conclude what effect pridopidine was truly having on HD-associated movement changes.
PRIDE-HD Trial
After this, the rights and ownership for the drug changed hands. The new owners, Teva Pharmaceuticals, wanted to test if a higher dose of pridopidine was needed to see a positive effect on HD movements. Enter the 2013 trial called PRIDE-HD.
In PRIDE-HD, a key goal was to see if pridopidine could improve total motor score – a robust collection of tests that assign a numerical value to movement symptoms associated with HD. Unfortunately, again, pridopidine failed to meet its primary endpoint and did not show an improvement in total motor score.
A change in direction
At this point, scientists went back to the drawing board to try and better understand the drug. They did more experiments and when they emerged, they had a new model for how pridopidine might improve brain health.
Now, the idea was that pridopidine might not just control HD-associated movements, but might modify disease course. This would mean pridopidine isn’t just treating the symptoms of HD, but is treating the disease itself – a huge difference.
New goal for PRIDE-HD
With this new theory, the drug developers added a few new goals mid-way through the PRIDE-HD study. The primary endpoint of testing movement was the same, and wasn’t met, but they also added a second test – total functional capacity, or TFC.
TFC is measured through a collection of tests that determine how well someone functions day-to-day. Things like the ability to hold a job, manage finances, and perform domestic chores. These abilities decline as HD progresses.
So did pridopidine improve TFC in the PRIDE-HD study? Kind of. Out of the 4 doses tested, only the lowest dose showed improvement on the TFC scale. These results were inconsistent, TFC wasn’t a primary endpoint of the trial, and there wasn’t enough data to draw a firm conclusion – so for the drug to have a chance of getting licensed, yet another trial would be needed.
Big conclusions were drawn – too big
Despite these inconsistent findings, Teva interpreted the results from PRIDE-HD to mean that the drug was slowing the progression of HD. This was a big message for the data that was generated. Quite frankly, too big of a message. This conclusion cannot be substantiated with the results from this trial.
Why not? It’s important to understand that improving or stabilizing TFC does not necessarily mean that something has slowed HD progression. For example, successful treatment of depression in a person with HD could enable them to resume work, improving that person’s TFC by 1 or 2 points – but not because the underlying progression of the disease has been slowed.
To be clear, a drug that improves the ability of people with HD to function would be fantastic. And a drug that slows the progression of HD would be super fantastic! But there is a clear distinction between those two.
Improvements in function cannot directly be interpreted as slowing HD progression – that requires much more solid evidence, from direct measures like MRI scans or biomarkers that can tell us whether something has rescued brain cells, or from much longer-term improvements in symptoms.
PROOF-HD Trial
Following the results from PRIDE-HD, pridopidine changed hands again, this time to Prilenia Therapeutics. Another trial was begun to test the ability of pridopidine to modify disease course of HD. This new Phase 3 trial began in 2020 under the name PROOF-HD.
This time, the primary endpoint was TFC, which it did not meet. People who took pridopidine in the PROOF-HD trial did not show any improvement in day-to-day functioning as measured by TFC. Again, this was a negative pridopidine trial. The trial also failed to meet its secondary endpoint, an overall assessment of HD severity called the composite Unified Huntington’s Disease Rating Scale (cUHDRS).
However, one would be hard-pressed to understand this, based on the headlines about the PROOF-HD trial. Again, there were controversial interpretations that have touted PROOF-HD as a success.
Drug interactions
Previous results had suggested an interaction between pridopidine and drugs that reduce dopamine activity in the brain. Dopamine is a chemical that helps control movement, memory, and mood. The interaction between pridopidine and these dopamine-altering drugs is perhaps unsurprising, given that pridopidine was originally designed to alter dopamine activity to help with HD movements.
Drugs that reduce dopamine activity in HD include commonly-used medications like tetrabenazine for chorea, or ‘neuroleptic’ drugs like olanzapine and risperidone, which are used to help control some of the most difficult symptoms of HD like aggression, impulsivity, paranoia, delusions, or suicidality. Physicians don’t take prescribing these medications lightly, and often do so to reduce the risk that a person with HD will harm themselves or others.
Prilenia decided, in advance, to do a ‘subgroup analysis’ of the PROOF-HD trial results in people who were not taking any of these dopamine-altering drugs. This group had only 79 people with HD compared with the full trial population of 499 people with HD. They reported that this subgroup had benefited from treatment on cUHDRS and one measure of cognition, but not the TFC.
A troubling narrative
Of the drug, Prilenia CEO Dr. Michael Hayden is quoted as saying, “Pridopidine has delivered consistent efficacy benefits across multiple key measures of HD”. We at HDBuzz do not agree that the data collected to date support this interpretation.
Pridopidine has been tested in more trials than any other drug in the HD landscape, and has consistently failed to deliver the benefits anticipated by the companies running the trials. Apparent improvements seen in one aspect of HD have not been replicated when tested in subsequent trials.
What if it’s true that pridopidine works in people not taking neuroleptics? That would be good news! But to make this claim and have the regulatory agencies believe it, another clinical trial would generally be needed, focusing exclusively on this group and enrolling enough people to test this robustly. This is a valid scientific hypothesis, and it’s reasonable to test it with such a trial.
However, that’s not what Prilenia are planning.
We were troubled by an announcement from a recent, non-peer-reviewed poster summary supported by Prilenia where the authors claimed to show links between neuroleptic treatment and the progression of HD. This is a very difficult thing to prove with existing data and statistics, since neuroleptics are generally given to people whose HD symptoms are worse, or whose HD is progressing more rapidly.
It is certainly important to study how different medications might impact HD progression. But when a company whose unapproved drug may rely on people not taking neuroleptics, starts supporting research into whether neuroleptics may be bad for people with HD, we worry about what conclusions HD families might draw, and we worry especially about people stopping medicines that are protecting themselves and others from harm.
Hope vs. hype
At HDBuzz, we’re less worried about the science around pridopidine – in every trial, the results are what you get, and continuing to test theories based on those results is reasonable, if the sponsor thinks there is a real effect to be found in a particular group of people. This process is what needs to be done until we get better drugs for HD.
What troubles us is the message being put out about pridopidine, downplaying the big things it has failed to do, and emphasizing less compelling findings in subgroups or individual endpoints. We worry that people from HD families – families like mine – could end up with a much more favorable impression than is warranted of pridopidine being the first disease-slowing drug for HD. Unfortunately, all the evidence so far does not support that hope.
Hope that a drug will work is useful – but only when that hope is grounded in truth.
We want people from HD families to participate in trials for drugs with a good chance of working. Drugs that have strong scientific reasoning and solid evidence to support asking people and families to make the sacrifice of time, effort, and risk.
We have the right to expect that trial results will be presented plainly and understandably. Trials that fail to meet their outcomes should not be presented as positive, and companies with vested interests should be extremely careful about commenting on how HD clinicians and their patients choose from currently available treatment options.
Getting an application accepted for review
Most recently, we’ve heard news from Prilenia about advancing pridopidine through the European regulatory agency, the EMA. Their recent press release titled, “Prilenia’s Pridopidine for Huntington’s Disease Accepted for European Marketing Authorisation Review” is notable for its use of the word “Accepted” considerably earlier than the word “Review”.
What the press release actually says is that Prilenia has put together the paperwork to ask the EMA to consider the results from pridopidine to date, and the EMA has accepted the submission of their application.
Getting applications accepted for consideration is a process that every approved drug goes through. But it’s also a process that every refused drug goes through. Applying to get into a university is very different from being accepted. We’re reliably informed by an expert familiar with EMA procedures that this step is not that big of a deal.
Most of the time, we don’t hear about when companies go through these minor steps. Press releases generally aren’t issued for these commonplace steps throughout the regulatory process. While it’s broadly good that Prilenia are ensuring the HD community gets regular updates on where they are in the regulatory chain, we want to make sure that the news isn’t causing undue hype. We’re more keen on seeing the full, peer-reviewed results of the PROOF-HD trial in a scientific journal.
What we know
Pridopidine has been tested in various clinical trials with various primary endpoints, all of which have sadly failed to be met. Regardless of the messaging being put out from Prilenia, thus far pridopidine has produced negative results from every major endpoint in every trial. Period.
We’re not even remotely happy about this: we love drugs that work, and we love trials that prove it. We even love negative trials – when the results are presented clearly, without spin, and give a scientifically-grounded path ahead, whether that’s planning another trial or calling it quits.
The goalpost for the intended use of pridopidine continues to shift: to control movements, to modify disease course, to modify disease course for those not on neuroleptics. It’s fantastic that Prilenia continue to study pridopidine in the lab! It’s in everyone’s best interest that researchers understand medicines from as many angles as possible. However, we need to make sure the intended use of a drug is shown through positive clinical trial results.
An application has been submitted to the EMA for consideration. So far, this doesn’t mean much. An application was submitted. It will be reviewed.
Seeking truth
Scientists are truth seekers. At HDBuzz, we believe researchers have a duty to accurately relay scientific findings to the patient communities seeking answers for a cure. For truth.
If hope that a drug will work becomes so blind that it turns to hype, something has gone wrong. Results from clinical trials, by design, are not subjective. Reporting results should also not be subjective. Messaging matters. Headlines matter. Ensuring that the patient community receives and understands the full, balanced truth matters.
The team at HDBuzz honestly does hope that pridopidine delivers on everything Prilenia says it does, and more! We all want a drug that makes a positive difference to people with HD, but right now there is only data from 79 people to support moving this drug forward. In our opinion, that isn’t sufficient for regulatory approval – time will tell whether the regulators have the same view or are persuaded otherwise.
Until we get there, HDBuzz will be here to report the truth and tease out the hope from the hype. We’re sorry if our take is disappointing, but we make no apology for keeping openness, frankness, and science at the heart of our reporting.